Subscribe to RSS

DOI: 10.1055/a-2081-9249
Recent Developments in Ultrasound-Promoted Aqueous-Mediated Greener Synthesis of Biologically Vital Indole Derivatives
Abstract
The extensive range of uses of N-heterocycles as potent bioactive motifs has attracted researchers to expand newer methods for their efficient synthesis. Particularly, indoles are widely known for their prevalent pharmacological properties. Green chemistry provides various synthetic tools viz. alternative energy resources, nonconventional solvents, nano-catalysts, etc. Modern strategies of using ultrasound as an alternative energy resource in organic synthesis has led to the development of environment friendly and cost-effective techniques. The chemical and mechanical effects of ultrasound waves impart significant enhancement in both stoichiometric and catalytic reactions. The exclusive physicochemical properties of water offer widespread utility for carrying out organic reactions in this medium. The aim of this review article is to provide an inclusive summary of the combined use of ultrasound and aqueous media for the facile synthesis of biologically vital indole derivatives.
1 Introduction
2 Synthesis of Biologically Vital Indoles
2.1 Spirocyclic Indoles
2.2 Non-spiro 3-Substituted Indoles
2.3 Miscellaneous Indole Syntheses
3 Conclusions
#
Biographical Sketches


Kanaram Choupra was born in Jaipur, Rajasthan, India. He earned his M. Sc. degree (2018) from the Indian institute of Technology Bombay, India. Currently, he is perusing a Ph. D. degree under the supervision of Prof. Meenakshi Jain from the Department of Chemistry, University of Rajasthan, Jaipur, India. His research focuses on ‘Facile synthesis of medicinally important indoles’.


Ashish Kumar Aheer was born in Ajmer, Rajasthan, India. He earned his M. Sc. degree (2017) from Samrat Prithviraj Chauhan Govt. College, Ajmer, Rajasthan, India. Currently, he is perusing a Ph. D. degree under the supervision of Prof. Meenakshi Jain from the Department of Chemistry, University of Rajasthan, Jaipur, India. His research focuses on ‘Synthesis of biologically important pyrazoles through greener methodologies’.


Prof. Anshu Dandia, FRSC was born in Ajmer, Rajasthan, India. She earned her M. Sc. (1978) and Ph. D. (1982) degrees from the University of Rajasthan, Jaipur, Rajasthan, India. Currently, she is working as Emeritus Scientist, UGC-BSR Faculty Fellow at the Department of Chemistry, University of Rajasthan, Jaipur, India. She also served as Head of Department of Chemistry and Director of P.G. School of Physical Sciences at the University of Rajasthan, Jaipur. Areas of her research interest include applications of nanotechnology in organic synthesis; heterocyclic and medicinal chemistry; development of environ-economic synthetic methodologies for value added materials; and microwave/ ultrasound/ visible light assisted organic synthesis.


Prof. Meenakshi Jain was born in Jaipur, Rajasthan, India. She earned her M. Sc. (1987) and Ph. D. (1992) degrees from the University of Rajasthan, Jaipur, Rajasthan, India. Currently, she is working as Professor and Head at the Department of Chemistry, University of Rajasthan, Jaipur, India. Prof. Jain’s research focuses on the Synthesis of medicinally important N-heterocyclic compounds, and on Green organic synthesis.


Dr. Amit Sharma was born in Nohar (Hanumangarh), Rajasthan, India. He earned his M. Sc. (2012) and Ph. D. (2017) degrees from University of Rajasthan, Jaipur, Rajasthan, India. Currently, he is working as an Assistant Professor at the Department of Chemistry, University of Rajasthan, Jaipur, India. Earlier, he was an Assistant Professor in Govt. PG College, Sri Ganganagar, Rajasthan, India. Dr. Sharma’s research focuses on the greener synthesis of biologically vital heterocyclic motifs, the synthesis of nano-materials and their applications in catalysis, and on ayurvedic drugs and nanotechnology.
Introduction
Green chemistry has provided facile, sustainable protocols with minimal or zero use of hazardous substances in the manufacturing and application of important chemicals.[1] One of the principles of green chemistry particularly focuses on the use of alternative energy resources to accomplish chemical reactions. The use of ultrasound waves (US) as an alternative energy source has grown considerably in various fields.[2] The acoustic cavitation process involved in US promoted methodologies generates huge amounts of energy due to high local temperature and pressure.[3] The sonochemical reactions proceed via the formation and adiabatic collapse of transitory cavitation bubbles in the liquid phase.[4a] [b] A lot of work has been done in this direction and it has been proved that US irradiation could facilitate the facile construction of biologically relevant organic moieties.[4c–i]
Nature’s solvent, water, has some fascinating structural and physicochemical features that lead to some particular intermolecular interactions such as hydrogen bonding, hydrophobic effects, and trans-phase interactions. These interactions lead to several energy changes during the course of a reaction.[5] However, the effective use of water as reaction medium requires incorporation of additives in order to homogenize mixtures of water and organic compounds.[6a] The use of US energy resource and aqueous media together can improve the applicability of both.[6b] The US waves travels through aqueous reaction medium and generates microcavitation bubbles. The cavitation bubbles are thought to act as micro reactors for the reactants. The reactant molecules enter into the micro bubbles and the instantaneous high local temperature and pressure produced during cavitations (due to formation and adiabatic collapse of cavitation bubbles) not only enhances the chances of reactants coming closer to each other but also lets the reactants overcome the potential energy barrier, required for a reaction to occur, very speedily (Figure [1]).[7] In this manner, ultrasound-promoted aqueous-mediated organic synthesis helps in achieving the goal of green synthesis.
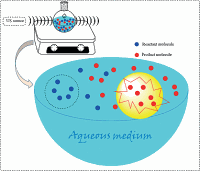

Most of the known synthetic and naturally occurring drugs and other bioactive compounds have a key heterocycle structural unit.[8] Furthermore, heterocycles are widely used in various fields such as dyestuffs, fluorescent materials, luminescent sensors, brightening agents, and analytical reagents.[9] They are also of great significance due to their wide utility as protecting groups, chiral auxiliaries, organocatalysts and ligands in asymmetric catalysts.[10] Particularly, the indole nucleus is associated with various biological activities viz. antibacterial, antifungal, antiviral, antitumor, anti-inflammatory, antidepressant, antimalarial, and anti-HIV agents. A large number of synthetic indoles have profound applications as pharmaceuticals and agrochemicals.[11] Representatives of bioactive indoles are depicted in Figure [2].


Therefore, the synthesis and functionalization of indoles has been an interesting object for researchers in the current epoch. Recently, extensive efforts have been undertaken to develop more efficient routes to synthesize biologically vital indole-based compounds.[12] This review focuses on the combined use of ultrasound and aqueous media for the facile synthesis of various important indole derivatives.
# 2
Synthesis of Biologically Vital Indoles
# 2.1
Spirocyclic Indoles
A library of spiro[indoline-3,4′-pyrazolo[3,4-b]pyridine]-2,6′(1′H)-diones 4a–p was synthesized by Bazgir and co-workers through a three-component reaction of 4-hydroxycoumarin, 1H-pyrazol-5-amines, and isatins in water under US irradiation using p-TSA as catalyst (Scheme [1]).[13] This one-pot protocol involves the formation of two C–C bonds and one C–O bond, and produces a series of spirooxindole-fused pyrazolopyridine derivatives. The authors performed the reaction at various temperatures (40, 50, and 60 °C), and found 60 °C to be a suitable temperature for the conversion of reactants into the desired product under ultrasonication in water.


Dandia and co-workers have done a lot of work on the synthesis of spirooxindoles and other indole derivatives. A library of spiro[indole-thiazolidinone] derivatives 8a–uu was synthesized by Dandia et al., via a US-promoted aqueous-mediated route. The approach involved the tandem reaction of isatins, 2-mercaptopropionic acid, and heterocyclic amines and proceeded in the presence of cetyltrimethylammonium bromide (CTAB) phase-transfer catalyst. A variety of heterocyclic cores including dimethyl-2-phenyl-pyrazol-3-one, triazole, benzimidazole, benzothiazole, and indazole, bearing a primary amino group were utilized as precursors in this protocol (Scheme [2]).[14] The surfactant CTAB in water enhanced the effect of US waves in accelerating the reaction, as it acts as an emulsifying agent and forms colloidal dispersion with organic substrates, consequently, rendering the mixture more homogeneous. Further, the authors summarized that the use of a high-intensity ultrasonic (HIU) direct immersion probe as US irradiation source provided better results in terms of yield and time than obtained with a low-intensity ultrasonic (LIU) cleaner.


Further, various spiro(indoline-3,4′-pyrano[2,3-c]pyrazole) derivatives 12a–l were synthesized through a three-component US-promoted reaction of substituted isatins, active methylene reagent, and 3-methyl-1-phenyl-2-pyrazolin-5-one in aqueous medium using cerium(IV) ammonium nitrate (CAN) as catalyst (Scheme [3]).[15] The acoustic cavitation stimulates the sonolysis and creation of bonds with the help of the Ce based catalyst.


Moreover, the authors also checked the antioxidant activities of the as-synthesized spiroindolinones and the data were compared with standard drug ascorbic acid. They used synthetic nitrogen-centered DPPH•, ABTS•+ and NO radicals as indicator compounds in the experimental studies. The authors found that the as-synthesized spiroindoline derivatives showed good radical scavenging abilities; however, their abilities were moderately lower than those of ascorbic acid. The combination of carboxylate group with N-benzyl substitution in the indole ring showed excellent DPPH• radical-scavenging activity.
The authors also proposed a plausible mechanism in this work (Scheme [4]). Accordingly, the acoustic cavitation played an important role in accelerating the CAN-catalyzed reaction through its induced shear forces and the jets produced near the surface of the vessel. The reaction involves formation of the isatiylidene malanonitrile intermediate and its further attack at the C-4 site of 3-methyl-1-phenyl-2-pyrazolin-5-one followed by intramolecular nucleophilic attack of the -OH group on the cyano moiety.


Another one-pot, three-component synthesis of spiro[indoline-3,4′-pyrano[2,3-c]pyrazole] and spiro[chromene-4,3′-indoline] derivatives 16a–l was achieved by Dandia and co-workers using ZnS nanoparticles as catalyst under ultrasonic irradiation in aqueous medium. Isatins, active methylene reagent, and 3-methyl-1-phenyl-2-pyrazolin-5-one or dimedone were used as precursor in this protocol (Scheme [5]).[16] The authors employed a variety of isatin substrates bearing electron-withdrawing and electron-releasing groups, and found that this protocol provided great yields with both.


Dandia and co-workers also explored the vital use of NaCl salt in water under ultrasonication for the efficient one-pot synthesis of spiro[chromene-4,3′-indolines] 20a–l via the reaction of isatins, active methylene component (malononitrile or ethylcyanoacetate), and dimedone (Scheme [6]).[17] All the reactions were performed at ambient temperature. The authors further developed the utility of NaCl and synthesized various spiro[indoline-3,4′-pyrano[2,3-c]pyrazole] derivatives through the same protocol using 3-methyl-1-phenyl-2-pyrazolin-5-one instead of dimedone. Besides producing a huge amount of energy, the acoustic cavitation effect here could contribute to the site-specific activation of substrate molecules by NaCl in water.
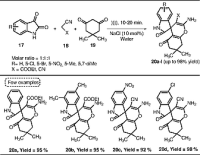

A catalyst-free aqueous-mediated multicomponent domino (MCR) protocol was also developed by Dandia et al. This method involves the chemo- and regio-selective synthesis of spiro[indoline-3,4′-pyrazolo[3,4-e][1,4]thiazepine]diones 25a–i via three-component reaction of isatin, 5-amino-3-methylpyrazole, and α-mercaptocarboxylic acid in water under US irradiation (Scheme [7]).[18] The reaction proceeded via Baylis–Hillman type adduct 24 intermediate formation. The authors interestingly explained the efficiency of water over other solvents used in this protocol under US irradiation by considering the I max (maximum cavitation intensity) and T Imax (the temperature at which I max is reached) parameters. Further, the authors tested the synthesized compounds for their α-amylase inhibition activity. Among the spiro[pyrazolo[3,4-e][1,4]thiazepine] derivatives synthesized in this work, compound 25c showed the best results.
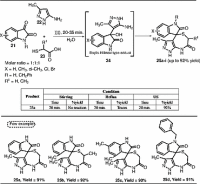

In the proposed mechanism, the authors well explained the plausible role of water in promoting the reaction. Water could create hydrogen bonds with C3-carbonyl functionality of isatin to a reasonable extent and increase the C3 electrophilicity of the isatin. Here, water works as a Brønsted acid and results in the rapid formation of Baylis–Hillman type adduct 24 (Scheme [8]). Further, the water can act as Brønsted base by connecting its oxygen site with the hydrogen of the thiol group and accelerate the Michael addition reaction of intermediate A with the thioacid, followed by dehydration.


Arya et al. reported the synthesis of spiro[indole-pyrido[3,2-e]thiazine] derivatives 29a–j via the reaction of various isatins with amine and 2-mercaptonicotinic acid catalyzed by ZSM-5-([MIM]+BF4 −) Brønsted acid ionic liquid catalyst system in water under US irradiation (Scheme [9]). The authors proved the effectiveness of US in aqueous medium over conventional and microwave irradiation methods. Further, they explained the particular role of water as reaction medium under ultrasonication in this reaction by giving the example of the formation of highly reactive radicals that are responsible for the rapid organic transformation.[19]


As proposed by the authors (Scheme [10]), the generation of imine diradical A in situ by the reaction of substituted isatin 26 and aromatic amines 27 while being subjected to sonication is the key step in the mechanism. Further, diradical intermediate A reacts with 2-mercaptonicotinic acid 28 to give the desired product via diradical intermediate B.


Various spiro[4H-pyrano[3,2-c]quinoline-4,3′-indoline]-2′,5(6H)-dione derivatives 33a–j were prepared by Holizadeh and Radmoghadam. They performed the reaction of 4-hydroxy-2H-quinolin-2-one, malononitrile or ethyl cyanoacetate and isatins in water assisted by US irradiation using piperidine as catalyst (Scheme [11]).[20] The authors also carried out the reaction without sonication in water and they observed that the reaction proceeded in 80 minutes while the use of US irradiation decreased the reaction time by 16 times. Probably the application of US increased the solvability of water to provide the better results. The authors also observed that the amidic N-H group of 4-hydroxy-2H-quinolone did not have any significant effect on the course of reaction, since N-substituted and unsubstituted quinolones participated similarly in the reaction.


According to the proposed mechanism, the reaction proceeds with the formation of intermediate isatylidenemalononitrile A by the condensation of isatin 30 and malononitrile 31. Then, 4-hydroxyquinolin-2(1H)-one 32 moiety attacks intermediate A in a Michael type addition manner, at its exocyclic C=C bond; this is then followed by an intramolecular cyclization to give desired product 33 (Scheme [12]).


The efficacy of US waves in organic synthesis has also been demonstrated by Naeimi et al. by providing a one-pot synthetic protocol for various tetrahydrospiro[chromene-4,3′-indoline] derivatives 37a–h using sulfonated chitosan-coated Fe3O4 nanoparticles (Fe3O4@CS-SO3H NPs) in aqueous media (Scheme [13]). Various isatins, malononitrile, and 1,3-dicarbonyl compounds were used as precursors in this reaction.[7b] The authors performed the experiment at a range of US frequencies and found 35 KHz to be optimal in terms of reaction yield and time parameters.


Hojati and co-workers developed a US-assisted, catalyst-free, room-temperature protocol for the synthesis of spiro[indoline-3,4′-pyrano[2,3-c]pyrazole] derivatives 42a–l via the four-component reaction of ethyl acetoacetate, hydrazine hydrate or phenylhydrazine, isatins, and malononitrile in aqueous media (Scheme [14]).[21] They proved the effective role of US in a noncatalyzed route over a catalyzed pathway. The reaction proceeded smoothly in just 5 minutes with isatin but required some more time in the case of substituted isatins.


A protocol involving the Knoevenagel–Michael cyclization of isatins, malononitrile and α-isotriocyanatoimide to afford various 3,3′-pyrrolidonyl-spirooxindoles 46a–p in water under US irradiation at room temperature has been reported by Miao et al. (Scheme [15]).[22] They explained that sonication is responsible for rapid dispersion of solids on the surface of water and, consequently, enhancement of contact between molecules.


The authors also proposed a mechanism (Scheme [16]) for the above protocol that involves the formation of dicyanoalkene intermediate A by Knoevenagel condensation of isatin 43 with malononitrile 44. In the next step, Michael addition of the Knoevenagel adduct A with the enolate intermediate B, formed from α-isothiocyanatoimide 45, to form intermediate C. This intermediate C undergoes an intramolecular cyclization to afford the desired product 46 via intermediate D.


Liju et al. reported a one-pot, four-component reaction of substituted phenylhydrazine, dialkyl acetylenedicarboxylate, substituted isatins, and malononitrile in water/ethanol medium catalyzed by l-proline (Scheme [17]). This ultrasound-promoted, room-temperature protocol provides an extensive range of spiro[indoline-3,4′-pyrano[2,3-c]pyrazole] derivatives 51a–r. Interestingly, the authors noted a change in the temperature of both the water bath and the reaction system during the reaction under ultrasonication.[23]


As proposed by authors (Scheme [18]), intermediate A, which is formed through the initial reaction of 47 and 48, undergoes enolization in the presence of l-proline to give B. Additionally, intermediate C is generated by the Knoevenagel condensation between 49 and 50. Further, l-proline catalyses the deprotonation of intermediate B and its Michael type addition to C to give intermediate D. This intermediate D produces the desired product 51 through intramolecular cyclization.




A series of spiro-oxindoles has been synthesized by Ghomi and co-workers in water using US as an energy resource at room temperature (Scheme [19]).[24] They performed one-pot multicomponent reaction of various isatins, dimethylacetylenedicarboxylate (DMAD), malononitrile, and various hydrazine hydrate or anilines in order to prepare spiro-oxindoles including spiropyrano[2,3-c]pyrazole carboxylate derivatives 56a–l and spiro[indoline-3,4′-pyridine] derivatives 58a–l. They used a supported molybdenum complex on cross-linked poly(1-aminopropyl-3-vinylimidazolium bromide) entrapped cobalt oxide nanoparticles (Co3O4@PPIL-Mo) as catalyst. In this report, the authors demonstrated and explained the synergistic effect of US waves, water, and active catalytic sites. US irradiation facilitated electron-transfer in the reaction environment of aqueous media. Further, the collapse of cavitation bubbles provided localized hotspots that helped the reaction to occur rapidly.[24]
# 2.2
Non-spiro 3-Substituted Indoles
A variety of bis(indolyl)methanes 61a–l were synthesized by Li et al. via electrophilic substitution reactions of indoles with aromatic carbonyl compounds in aqueous medium under ultrasound irradiation (Scheme [20]). This reaction was catalyzed by aminosulfonic acid.[25] Since the reaction involves attack of an electrophile on the indole ring, the reaction provided better results with aromatic aldehydes bearing electron-withdrawing substituents, as expected.


As per the proposed reaction mechanism (Scheme [21]), the protic acid activates the carbonyl functionality and the produced electrophile causes an electrophilic substitution reaction at the C3 carbon of indole 59. The formed intermediate A undergoes dehydration to give intermediate B. Further, intermediate B is activated by a proton and serves as an electrophile to attack a second molecule of indole to form the desired product 61.


The same research group also reported another improved methodology for synthesizing bis(indolyl)methanes 64a–m. Here, they have used dodecylbenzenesulfonic acid (ABS) as an effective catalyst for the greener synthesis. In this work they also demonstrated the combined effect of US irradiation and water for the facile synthesis (Scheme [22]).[26]


Gill and co-workers prepared a variety of bis(indol-3-yl)methanes 67a–n by the one-pot reaction of indole and various aldehydes in water using ultrasound irradiation at ambient temperature. They used 1-hexenesulphonic acid sodium salt as catalyst (Scheme [23]).[27] The authors showed that 1-hexenesulphonic acid sodium salt liberates the corresponding acid when dissolved in water during the course of the reaction and catalyzes the reaction to afford the desired product under US irradiation.


Khorshidi and Tabatabaeian have reported the ultrasound promoted synthesis of 3-indolyl-3-hydroxyoxindoles 70a–k in aqueous media by performing the reaction of isatins and indoles catalyzed by Fe(III) homogeneous catalyst. They reported that all products were solely monoindolylated isatins not 3,3-di-3-indolylindolin-2-ones (Scheme [24]).[28] The US waves helps in generating fine emulsions and enhances mass transfer in aqueous medium. The authors also checked the effect of US power (taking the frequency constant) on reaction commencement and observed that an increase in US power from 20 to 100% (from 92 to 460 W cm–2, respectively) resulted in a decrease in reaction time. At high power, the cavitation process is maximized and reactants become distributed throughout the reaction mixture effectively, which results in completion of reaction in shorter time.


Further, Li and co-workers demonstrated the combined effect of US and aqueous media for the one-pot Mannich reaction of secondary amine, formaldehyde, and indole or N-methylindole giving Mannich bases 74a–i related to gramine (Scheme [25]).[29] Further, the authors examined the role of US frequency on this reaction protocol and found better results in case of 25 kHz US frequency source than with a 40 kHz frequency source. The lower frequency irradiation can produce better cavitation than higher frequency.


The Amrollahi research group reported the synthesis of bis(indole) derivatives 77a–l by the reaction of indole with various electron-deficient alkenes in aqueous media under ultrasonication using 12-tungstophosphoric acid (H3PW12O40) as catalyst (Scheme [26]).




The expected bis(indole) derivatives were obtained with various 2-benzylidenemalononitrile and 3-(phenyl)acrylates,[30] but when 2-(pyridylmethylene)malononitriles and 3-(pyridyl)acrylates were used as electron-deficient alkenes, only the corresponding indolyl derivatives 80a–f were formed (Scheme [27]). The authors presented a reason for this result. Possibly, in the presence of H3PW12O40 acid catalyst, the oxidative dehydrogenation of 2-((indolyl)(pyridyl)-methylene)malononitriles and of 3-(indolyl)(pyridyl)acrylates catalyst is less reactive than 2-((aryl)(indolyl)methyl)malonitriles and 3-(aryl)(indolyl)acrylates, respectively, which stops the reaction up to indolyls.
Further, in another work, the authors demonstrated a one-pot, four-component procedure for condensing indole, aldehydes, and active methylene compounds in aqueous media to give bis(indole) derivatives 84a–l under ultrasonication using 12-tungstophosphoric acid as catalyst (Scheme [28]).[31]


Here also, bis(indole) derivatives were obtained in case of various benzaldehydes but only mono-substituted products 88a–l were obtained with various pyridinecarboxaldehydes (Scheme [29]).


Kaur et al. explored the utility of US and water for the efficient synthesis of a variety of 3-amino alkylated indoles 92a–y (Scheme [30]). They performed the reaction of aromatic aldehydes and aniline derivatives in aqueous media using US as an alternative energy resource and PLA (polylactic acid) grafted ZnO nanoparticles as catalyst.[32]


Yonemitsu condensation for the preparation of 3-indole derivatives 96a–q was performed by Lu and co-workers (Scheme [31]). They explored the use of US irradiation for facile synthesis in aqueous glycerol using indoles and Meldrum’s acid as precursor at room temperature without catalyst.[33] The authors reported that aliphatic aldehydes showed lower reactivity in this reaction than aromatic aldehydes. Particularly for aromatic aldehydes, the ortho-substituted aromatic aldehydes gave lower yields, probably due to the steric hindrance at the ortho position.


Nikpassand et al. utilized various pyrazolecarbaldehydes and developed a protocol for their reaction with indole to give bis(indolyl)methanes 99a–j under US irradiation using water as reaction medium (Scheme [32]).[34] Pyrazole carbaldehydes bearing electron-withdrawing groups showed higher reactivity than their electron-releasing counterparts.


Aqueous mediated synthesis of bis(indolyl)methanes 102a–n was reported by Kasar and Thopate using US as an alternative energy resource catalyzed by malic acid organocatalyst (Scheme [33]). This ambient-temperature protocol involved the reaction of indole and various aromatic aldehydes.[35]


A series of bis(indolyl)methane derivatives 105a–o were prepared by the Deshmukh research group using pyruvic acid catalyst in water under ultrasonication (Scheme [34]).[36] The authors synthesized 15 compounds by using this protocol and they compared all the US-promoted reactions with the conventional method. They found that while synthesizing all these 15 bis(indolyl)methane products, the US-promoted methodology had advantages over conventional approach in terms of both reaction time and yields.


The authors also proposed a reaction mechanism involving the pyruvic acid as protic acid catalyst (Scheme [35]). Essentially, the carbonyl functionality is activated by the protic acid, which facilitates the electrophilic attack of protonated carbonyl A at C3 carbon of indole 103 and further generation of condensed intermediate B. The desired product 105 is formed by the attack of intermediate B on a second molecule of indole.


Miscellaneous Indole Syntheses
Various spiro[indole-oxathiolanes] 108a–f were prepared by Dandia and co-workers in aqueous media under US by the reaction of spiro[indole-3,2′-oxiranes] with thioacetamide using LiBr as catalyst (Scheme [36]).[37] Here, US energy proved to be a better source than the microwave source. The authors also explained the role of US in heterogeneous reaction conditions. They emphasized that both mechanical and chemical effects of sonochemical cavitation are responsible for the acceleration of reaction rate.


The same research group also reported the diastereoselective synthesis of fluorine-containing spiro[indole-3,2′-oxirane]-3′-benzoyl-2(1H)-ones 110a–g by the epoxidation of 3-aroylmethylene indole-2-one in aqueous medium using US as energy resource (Scheme [37]).[38]


The authors also screened the synthesized compounds for antimicrobial and antioxidant activities. They showed that Gram negative bacteria are more susceptible to the as-synthesized compounds than Gram positive organisms. In general, all the synthesized compounds showed moderate to good activity against all the bacteria. Particularly, compound 110d showed excellent activity against E. coli. The synthesized spiro[indole-3,2′-oxiranes] derivatives bearing halogen substituents also showed greater antioxidant properties with respect to NO and DPPH methods.[38]
Ramana and co-workers reported the synthesis of dihydropyrano[2,3-e]indole derivatives 114a–h via the three-component reaction of aldehydes, malononitrile, and 1-tosyl-1H-indol-4-ol under ultrasonication in water/ethanol solvent system. Barium titanate nanoparticles (BaTiO3 NPs) were used as catalyst to catalyze this reaction (Scheme [38]).[39] The reaction proceeded more efficiently using US horn than that in a sonication bath.


As proposed by the authors in the plausible mechanism (Scheme [39]), the metallic part (M n+) of the metal oxides acts as a Lewis acid whereas oxide (O2–) behaves as a base. The reaction involves initial Knoevenagel condensation of aldehyde and malononitrile, both activated by BaTiO3 NPs, resulting in the formation of intermediate A. This intermediate A undergoes Michael-type addition with 1-tosyl-1H-indol-4-ol to give intermediate B. Then, intermediate B undergoes Thorpe–Ziegler type cyclization, promoted by both the Lewis acidic and basic sites of BaTiO3 NPs, followed by tautomerization to give the desired product 114.


A variety of hydrazine carboxamides 117a–l were synthesized by Ahsan et al. in water-glycerol system under US irradiation (Scheme [40]). Isatin and various N-(substituted phenyl)hydrazine carboxamides were used as starting materials in this protocol.[40] The best results were obtained when water-glycerol (3:2) was used as a reaction medium under ultrasonication. Further, the authors investigated the synthesized compounds for in vitro anticancer activity against nine separate panels of 60 cancer cell lines according to NCI US protocols. On the basis of structure–activity relationship studies, it was concluded that the compound with 4-chloro substitution on the phenyl ring showed excellent anticancer activity.[40]


#
# 3
Conclusions
The acoustic cavitation in ultrasound promoted reactions generates a large amount of local heat and pressure that can assist in rapid conversion of reactants into products. This accelerated conversion can lead to highly selective synthesis in terms of regio-, chemo-, and stereoselectivity. Water is one of the safest solvents used in green reaction processes. The beneficial effects of these two, alternative US energy and Nature’s solvent water, are enhanced when used together. This combined strategy has been widely explored for the facile synthesis of biologically important indole derivatives. In this review article, we have collected the recently reported synthetic methodologies related to synthesis of indoles, including spiro-, non-spiro-, and other indole-based molecules, in aqueous medium under ultrasonication. Although, there is rapidly developing interest in this direction, some points remain to be addressed: (1) There is a large scope for work in the direction of enantio-selective synthesis of bis(indolyl)-derivatives in aqueous medium under ultrasonication. (2) New paths may be made possible for the utilization of two-dimensional carbon materials as catalytic supports by graphene nanosheets due to their huge specific surface area and presence of reactive oxygen functional groups. These materials can also be explored for the synthesis of various spiro- and non-spiro indole derivatives under US in water. (3) A lot of work can also be done on analyzing the bioactivities of newer indole derivatives synthesized under US in water.
We sincerely hope that this review article will provide comprehensive information on the synthesis of indole derivatives in aqueous medium under ultrasonication, and open new doors for the further development of related processes.
#
#
Conflict of Interest
The authors declare no conflict of interest.
Acknowledgment
The authors are grateful to the Department of Chemistry, University of Rajasthan, Jaipur, India, for providing the necessary facilities.
-
References
- 1a Ferrazzano L, Catani M, Cavazzini A, Martelli G, Corbisiero D, Cantelmi P, Fantoni T, Mattellone A, De Luca C, Felletti S, Cabri W, Tolomelli A. Green Chem. 2022; 24: 975
- 1b Zimmerman JB, Anastas PT, Erythropel HC, Leitner W. Science 2020; 367: 397
- 1c Anastas P, Eghbali N. Chem. Soc. Rev. 2010; 39: 301
- 2a Banerjee B. Ultrason. Sonochem. 2017; 35: 1
- 2b Puri S, Kaur B, Parmar A, Kumar H. Curr. Org. Chem. 2013; 17: 1790
- 2c Baig RB. N, Varma RS. Chem. Soc. Rev. 2012; 41: 1559
- 3a Das P, Bhattacharyya SK, Banerji P, Das NC. Nano-Struct. Nano-Objects 2021; 25: 100641
- 3b Zhao S, Yao C, Zhang Q, Chen G, Yuan Q. Chem. Eng. J. 2019; 374: 68
- 3c Muthupandian AK. Ultrason. Sonochem. 2011; 18: 864
- 4a Pagadala R, Kasi V, Shabalala NG, Jonnalagadda SB. Arabian J. Chem. 2022; 15: 103544
- 4b Patil R, Bhoir P, Deshpande P, Wattamwar T, Shirude M, Chaskar P. Ultrason. Sonochem. 2013; 20: 1327
- 4c Patil R, Bhoir P, Deshpande P, Wattamwar T, Shirude M, Chaskar P. Ultrason. Sonochem. 2013; 20: 1327
- 4d Banerjee B. Aust. J. Chem. 2017; 70: 872
- 4e Banerjee B. Ultrason. Sonochem. 2017; 35: 1
- 4f Kaur N. Synth. Commun. 2018; 48: 1235
- 4g Kaur G, Sharma A, Banerjee B. ChemistrySelect 2018; 3: 5283
- 4h Banerjee B. ChemistrySelect 2019; 4: 2484
- 4i Sharma A, Priya A, Kaur M, Singh A, Kaur G, Banerjee B. Synth. Commun. 2021; 51: 3209
- 5a Gawande MB, Bonifacio VD. B, Luque R, Brancoa PS, Varma RS. Chem. Soc. Rev. 2013; 42: 5522
- 5b Kommi DN, Kumar D, Seth K, Chakraborti AK. Org. Lett. 2013; 15: 1158
- 5c Butler RN, Coyne AG. Chem. Rev. 2010; 110: 6302
- 5d Leadbeater NE. Chem. Commun. 2005; 2881
- 6a Cortes-Clerget M, Yu J, Kincaid JR. A, Walde P, Gallou F, Lipshutz BH. Chem. Sci. 2021; 12: 4237
- 6b Banerjee B. J. Serb. Chem. Soc. 2017; 82: 755
- 7a Zhaoa S, Yao C, Dong Z, Chen G, Yuan Q. Particuology 2020; 48: 88
- 7b Naeimi H, Lahouti S. J. Iran. Chem. Soc. 2018; 15: 2017
- 7c Yusof NS. M, Babgi B, Aksu M, Madhavan J, Muthupandian AK. Ultrason. Sonochem. 2016; 29: 568
- 8a Martins P, Jesus J, Santos S, Raposo LR, Roma-Rodrigues C, Baptista PV, Fernandes AR. Molecules 2015; 20: 16852
- 8b Baumann M, Baxendale IR. Beilstein J. Org. Chem. 2013; 9: 2265
- 8c Dua R, Shrivastava S, Sonwane SK, Srivastava SK. Adv. Biol. Res. 2011; 5: 120
- 8d Baumann M, Baxendale IR, Ley SV, Nikbin N. Beilstein J. Org. Chem. 2011; 7: 442
- 8e Sharom MS, Miles JR. W, Harris CR, McEwen FL. Water Res. 1980; 14: 1095
- 9a Khan S, Quraishi MA. Arabian J. Sci. Eng. 2010; 35: 73
- 9b Behera GB, Behera PK, Mishra BK. J. Surf. Sci. Technol. 2007; 23: 1
- 9c Steingruber E. Indigo and Indigo Colorants. In Ullmann’s Encyclopedia of Industrial Chemistry. Wiley-VCH; Weinheim: 2004
- 9d Darzynkiewicz Z. Methods Cell Biol. 1990; 33: 285
- 10a Demirel N, Haber J, Ivlev SI, Meggers E. Organometallics 2022; 41: 3852
- 10b Lizza JR, Bremerich M, McCabe SR, Wipf P. Org. Lett. 2018; 20: 6760
- 10c Li QZ, Zeng R, Fan Y, Liu YQ, Qi T, Zhang X, Li JL. Angew. Chem. 2022; 61: e202116629
- 10d Diez-Gonzalez S, Marion N, Nolan SP. Chem. Rev. 2009; 109: 3612
- 11a Sravanthi TV, Manju SL. Eur. J. Pharm. Sci. 2016; 91: 1
- 11b Yan W, Zhao SS, Ye YH, Zhang YY, Zhang Y, Xu JY, Yin SM, Tan RX. J. Nat. Prod. 2019; 82: 2132
- 11c Palmisanoa G, Penonia A, Sistia M, Tibilettia F, Tollaria S, Nicholas KM. Curr. Org. Chem. 2010; 14: 2409
- 12a Zhang H.-H, Shi F. Acc. Chem. Res. 2022; 55: 2562
- 12b Nasri S, Bayat M, Miankooshki FR, Samet NH. Mol. Diversity 2022; 26: 3411
- 12c Tu M.-S, Chen K.-W, Wu P, Zhang Y.-C, Liu X.-Q, Shi F. Org. Chem. Front. 2021; 8: 2643
- 12d Zhang Y.-C, Jiang F, Shi F. Acc. Chem. Res. 2020; 53: 425
- 12e Mei G.-J, Shi F. Chem. Commun. 2018; 54: 6607
- 12f Yan LJ, Wang YC. ChemistrySelect 2016; 1: 6948
- 13 Bazgir A, Ahadi S, Ghahremanzadeh R, Khavasi HR, Mirzaei P. Ultrason. Sonochem. 2010; 17: 447
- 14 Dandia A, Singh R, Bhaskaran S, Samant SD. Green Chem. 2011; 13: 1852
- 15 Dandia A, Saini D, Bhaskaran S, Saini DK. Med. Chem. Res. 2014; 23: 725
- 16 Dandia A, Parewa V, Jain AK, Rathore KS. Green Chem. 2011; 13: 2135
- 17 Dandia A, Jain AK, Bhati DS. Synth. Commun. 2011; 41: 2905
- 18 Dandia A, Singh R, Joshi J, Maheshwari S, Soni P. RSC Adv. 2013; 3: 18992
- 19 Arya K, Rawat DS, Sasai H. Green Chem. 2012; 14: 1956
- 20 Gholizadeh S, Radmoghadam K. Orient. J. Chem. 2013; 29: 1637
- 21 Hojati SF, Mohamadi S. Org. Prep. Proced. Int. 2020; 52: 304
- 22 Yu CB, Lyu H, Cai Y, Miao XY, Miao ZW. RSC Adv. 2013; 3: 18857
- 23 Liju W, Ablajan K, Jun F. Ultrason. Sonochem. 2015; 22: 113
- 24 Elyasi Z, Ghomi JS, Najafi GR. Ultrason. Sonochem. 2021; 75: 105614
- 25 Li JT, Dai HG, Xu WZ, Li TS. Ultrason. Sonochem. 2006; 13: 24
- 26 Li JT, Sun MX, He GY, Xu XY. Ultrason. Sonochem. 2011; 18: 412
- 27 Joshi RS, Mandhane PG, Diwakar SD, Gill CH. Ultrason. Sonochem. 2010; 17: 298
- 28 Khorshidi A, Tabatabaeian K. J. Serb. Chem. Soc. 2011; 76: 1347
- 29 Li JT, Sun SF, Sun MX. Ultrason. Sonochem. 2011; 18: 42
- 30 Rahimi S, Amrollahi MA, Kheilkordi Z. C. R. Chim. 2015; 18: 558
- 31 Amrollahi MA, Kheilkordi Z. J. Iran. Chem. Soc. 2016; 13: 925
- 32 Shaikh T, Sharma A, Kaur H. ChemistrySelect 2019; 4: 245
- 33 Lu CW, Wang JJ, Liu YH, Shan WJ, Sun Q, Shi L. Res. Chem. Intermed. 2017; 43: 943
- 34 Nikpassand M, Fekri LZ, Nabatzadeh M. Synth. Commun. 2017; 47: 29
- 35 Kasar SB, Thopate SR. Curr. Green Chem. 2018; 5: 177
- 36 Deshmukh SR, Nalkar AS, Thopate SR. Polycyclic Aromat. Compd. 2022; 42: 6501
- 37 Dandia A, Singh R, Bhaskaran S. Ultrason. Sonochem. 2010; 17: 399
- 38 Dandia A, Singh R, Bhaskaran S. Ultrason. Sonochem. 2011; 18: 1113
- 39 Shaikh S, Rasal S, Ramana MM. V. React. Kinet., Mech. Catal. 2021; 133: 405
- 40 Ali A, Ali A, Tahir A, Bakht MA, Ahsan MJ. J. Enzyme Inhib. Med. Chem. 2022; 37: 135
Corresponding Authors
Publication History
Received: 05 January 2023
Accepted after revision: 20 April 2023
Accepted Manuscript online:
26 April 2023
Article published online:
24 May 2023
© 2023. The Author(s). This is an open access article published by Thieme under the terms of the Creative Commons Attribution License, permitting copying and reproduction so long as the original work is given appropriate credit. Contents may not be used for commercial purposes or adapted, remixed, transformed or built upon. (https://creativecommons.org/licenses/by/4.0/)
Georg Thieme Verlag KG
Rüdigerstraße 14, 70469 Stuttgart, Germany
-
References
- 1a Ferrazzano L, Catani M, Cavazzini A, Martelli G, Corbisiero D, Cantelmi P, Fantoni T, Mattellone A, De Luca C, Felletti S, Cabri W, Tolomelli A. Green Chem. 2022; 24: 975
- 1b Zimmerman JB, Anastas PT, Erythropel HC, Leitner W. Science 2020; 367: 397
- 1c Anastas P, Eghbali N. Chem. Soc. Rev. 2010; 39: 301
- 2a Banerjee B. Ultrason. Sonochem. 2017; 35: 1
- 2b Puri S, Kaur B, Parmar A, Kumar H. Curr. Org. Chem. 2013; 17: 1790
- 2c Baig RB. N, Varma RS. Chem. Soc. Rev. 2012; 41: 1559
- 3a Das P, Bhattacharyya SK, Banerji P, Das NC. Nano-Struct. Nano-Objects 2021; 25: 100641
- 3b Zhao S, Yao C, Zhang Q, Chen G, Yuan Q. Chem. Eng. J. 2019; 374: 68
- 3c Muthupandian AK. Ultrason. Sonochem. 2011; 18: 864
- 4a Pagadala R, Kasi V, Shabalala NG, Jonnalagadda SB. Arabian J. Chem. 2022; 15: 103544
- 4b Patil R, Bhoir P, Deshpande P, Wattamwar T, Shirude M, Chaskar P. Ultrason. Sonochem. 2013; 20: 1327
- 4c Patil R, Bhoir P, Deshpande P, Wattamwar T, Shirude M, Chaskar P. Ultrason. Sonochem. 2013; 20: 1327
- 4d Banerjee B. Aust. J. Chem. 2017; 70: 872
- 4e Banerjee B. Ultrason. Sonochem. 2017; 35: 1
- 4f Kaur N. Synth. Commun. 2018; 48: 1235
- 4g Kaur G, Sharma A, Banerjee B. ChemistrySelect 2018; 3: 5283
- 4h Banerjee B. ChemistrySelect 2019; 4: 2484
- 4i Sharma A, Priya A, Kaur M, Singh A, Kaur G, Banerjee B. Synth. Commun. 2021; 51: 3209
- 5a Gawande MB, Bonifacio VD. B, Luque R, Brancoa PS, Varma RS. Chem. Soc. Rev. 2013; 42: 5522
- 5b Kommi DN, Kumar D, Seth K, Chakraborti AK. Org. Lett. 2013; 15: 1158
- 5c Butler RN, Coyne AG. Chem. Rev. 2010; 110: 6302
- 5d Leadbeater NE. Chem. Commun. 2005; 2881
- 6a Cortes-Clerget M, Yu J, Kincaid JR. A, Walde P, Gallou F, Lipshutz BH. Chem. Sci. 2021; 12: 4237
- 6b Banerjee B. J. Serb. Chem. Soc. 2017; 82: 755
- 7a Zhaoa S, Yao C, Dong Z, Chen G, Yuan Q. Particuology 2020; 48: 88
- 7b Naeimi H, Lahouti S. J. Iran. Chem. Soc. 2018; 15: 2017
- 7c Yusof NS. M, Babgi B, Aksu M, Madhavan J, Muthupandian AK. Ultrason. Sonochem. 2016; 29: 568
- 8a Martins P, Jesus J, Santos S, Raposo LR, Roma-Rodrigues C, Baptista PV, Fernandes AR. Molecules 2015; 20: 16852
- 8b Baumann M, Baxendale IR. Beilstein J. Org. Chem. 2013; 9: 2265
- 8c Dua R, Shrivastava S, Sonwane SK, Srivastava SK. Adv. Biol. Res. 2011; 5: 120
- 8d Baumann M, Baxendale IR, Ley SV, Nikbin N. Beilstein J. Org. Chem. 2011; 7: 442
- 8e Sharom MS, Miles JR. W, Harris CR, McEwen FL. Water Res. 1980; 14: 1095
- 9a Khan S, Quraishi MA. Arabian J. Sci. Eng. 2010; 35: 73
- 9b Behera GB, Behera PK, Mishra BK. J. Surf. Sci. Technol. 2007; 23: 1
- 9c Steingruber E. Indigo and Indigo Colorants. In Ullmann’s Encyclopedia of Industrial Chemistry. Wiley-VCH; Weinheim: 2004
- 9d Darzynkiewicz Z. Methods Cell Biol. 1990; 33: 285
- 10a Demirel N, Haber J, Ivlev SI, Meggers E. Organometallics 2022; 41: 3852
- 10b Lizza JR, Bremerich M, McCabe SR, Wipf P. Org. Lett. 2018; 20: 6760
- 10c Li QZ, Zeng R, Fan Y, Liu YQ, Qi T, Zhang X, Li JL. Angew. Chem. 2022; 61: e202116629
- 10d Diez-Gonzalez S, Marion N, Nolan SP. Chem. Rev. 2009; 109: 3612
- 11a Sravanthi TV, Manju SL. Eur. J. Pharm. Sci. 2016; 91: 1
- 11b Yan W, Zhao SS, Ye YH, Zhang YY, Zhang Y, Xu JY, Yin SM, Tan RX. J. Nat. Prod. 2019; 82: 2132
- 11c Palmisanoa G, Penonia A, Sistia M, Tibilettia F, Tollaria S, Nicholas KM. Curr. Org. Chem. 2010; 14: 2409
- 12a Zhang H.-H, Shi F. Acc. Chem. Res. 2022; 55: 2562
- 12b Nasri S, Bayat M, Miankooshki FR, Samet NH. Mol. Diversity 2022; 26: 3411
- 12c Tu M.-S, Chen K.-W, Wu P, Zhang Y.-C, Liu X.-Q, Shi F. Org. Chem. Front. 2021; 8: 2643
- 12d Zhang Y.-C, Jiang F, Shi F. Acc. Chem. Res. 2020; 53: 425
- 12e Mei G.-J, Shi F. Chem. Commun. 2018; 54: 6607
- 12f Yan LJ, Wang YC. ChemistrySelect 2016; 1: 6948
- 13 Bazgir A, Ahadi S, Ghahremanzadeh R, Khavasi HR, Mirzaei P. Ultrason. Sonochem. 2010; 17: 447
- 14 Dandia A, Singh R, Bhaskaran S, Samant SD. Green Chem. 2011; 13: 1852
- 15 Dandia A, Saini D, Bhaskaran S, Saini DK. Med. Chem. Res. 2014; 23: 725
- 16 Dandia A, Parewa V, Jain AK, Rathore KS. Green Chem. 2011; 13: 2135
- 17 Dandia A, Jain AK, Bhati DS. Synth. Commun. 2011; 41: 2905
- 18 Dandia A, Singh R, Joshi J, Maheshwari S, Soni P. RSC Adv. 2013; 3: 18992
- 19 Arya K, Rawat DS, Sasai H. Green Chem. 2012; 14: 1956
- 20 Gholizadeh S, Radmoghadam K. Orient. J. Chem. 2013; 29: 1637
- 21 Hojati SF, Mohamadi S. Org. Prep. Proced. Int. 2020; 52: 304
- 22 Yu CB, Lyu H, Cai Y, Miao XY, Miao ZW. RSC Adv. 2013; 3: 18857
- 23 Liju W, Ablajan K, Jun F. Ultrason. Sonochem. 2015; 22: 113
- 24 Elyasi Z, Ghomi JS, Najafi GR. Ultrason. Sonochem. 2021; 75: 105614
- 25 Li JT, Dai HG, Xu WZ, Li TS. Ultrason. Sonochem. 2006; 13: 24
- 26 Li JT, Sun MX, He GY, Xu XY. Ultrason. Sonochem. 2011; 18: 412
- 27 Joshi RS, Mandhane PG, Diwakar SD, Gill CH. Ultrason. Sonochem. 2010; 17: 298
- 28 Khorshidi A, Tabatabaeian K. J. Serb. Chem. Soc. 2011; 76: 1347
- 29 Li JT, Sun SF, Sun MX. Ultrason. Sonochem. 2011; 18: 42
- 30 Rahimi S, Amrollahi MA, Kheilkordi Z. C. R. Chim. 2015; 18: 558
- 31 Amrollahi MA, Kheilkordi Z. J. Iran. Chem. Soc. 2016; 13: 925
- 32 Shaikh T, Sharma A, Kaur H. ChemistrySelect 2019; 4: 245
- 33 Lu CW, Wang JJ, Liu YH, Shan WJ, Sun Q, Shi L. Res. Chem. Intermed. 2017; 43: 943
- 34 Nikpassand M, Fekri LZ, Nabatzadeh M. Synth. Commun. 2017; 47: 29
- 35 Kasar SB, Thopate SR. Curr. Green Chem. 2018; 5: 177
- 36 Deshmukh SR, Nalkar AS, Thopate SR. Polycyclic Aromat. Compd. 2022; 42: 6501
- 37 Dandia A, Singh R, Bhaskaran S. Ultrason. Sonochem. 2010; 17: 399
- 38 Dandia A, Singh R, Bhaskaran S. Ultrason. Sonochem. 2011; 18: 1113
- 39 Shaikh S, Rasal S, Ramana MM. V. React. Kinet., Mech. Catal. 2021; 133: 405
- 40 Ali A, Ali A, Tahir A, Bakht MA, Ahsan MJ. J. Enzyme Inhib. Med. Chem. 2022; 37: 135










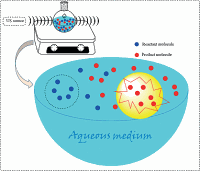













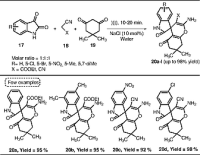

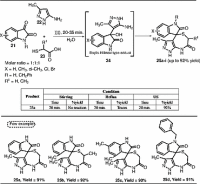


































































